ABSTRACT
Background: The intake of whey, compared with casein and soy protein intakes, stimulates a greater acute response of muscle protein synthesis (MPS) to protein ingestion in rested and exercised muscle.
Objective: We characterized the dose-response relation of postabsorptive rates of myofibrillar MPS to increasing amounts of whey protein at rest and after exercise in resistance-trained, young men.
Design: Volunteers (n = 48) consumed a standardized, high-protein (0.54 g/kg body mass) breakfast. Three hours later, a bout of unilateral exercise (8 × 10 leg presses and leg extensions; 80% one-repetition maximum) was performed. Volunteers ingested 0, 10, 20, or 40 g whey protein isolate immediately (∼10 min) after exercise. Postabsorptive rates of myofibrillar MPS and whole-body rates of phenylalanine oxidation and urea production were measured over a 4-h postdrink period by continuous tracer infusion of labeled [13C6] phenylalanine and [15N2] urea.
Results: Myofibrillar MPS (mean ± SD) increased (P < 0.05) above 0 g whey protein (0.041 ± 0.015%/h) by 49% and 56% with the ingestion of 20 and 40 g whey protein, respectively, whereas no additional stimulation was observed with 10 g whey protein (P > 0.05). Rates of phenylalanine oxidation and urea production increased with the ingestion of 40 g whey protein.
Conclusions: A 20-g dose of whey protein is sufficient for the maximal stimulation of postabsorptive rates of myofibrillar MPS in rested and exercised muscle of ∼80-kg resistance-trained, young men. A dose of whey protein >20 g stimulates amino acid oxidation and ureagenesis. This trial was registered at http://www.isrctn.org/ as ISRCTN92528122.
Topic:
- oxidation
- amino acids
- exercise
- muscle proteins
- phenylalanine
- leg
- urea
- resistive exercise
- ingestion
- whey proteins
- infusion procedures
Issue Section:
INTRODUCTION
Muscle mass is critical for human health, physical activity, and athletic performance (1). The metabolic basis that underlies increased muscle mass is the net muscle protein balance. Hyperaminoacidemia from protein ingestion increases net muscle protein balance, primarily because of increased muscle protein synthesis (MPS)4, with a lesser contribution from decreased muscle protein breakdown (2, 3). To increase or maintain muscle mass, it is important to investigate the optimal dose of protein for the maximal stimulation of MPS in exercised and rested muscle.
Studies have investigated the optimal dose of ingested protein for maximal stimulation of MPS in exercised and rested muscle of old and young men (4–8). In older adults, 40 g whey (8) or soy protein (9) stimulated a greater postexercise response of MPS than did 20 g protein. Likewise, mean MPS rates were higher with the ingestion of 35 (6) or 40 (8) compared with 20 g whey protein in rested muscle of older adults. Therefore, a 40-g dose of protein [equivalent to ∼20 g essential amino acids (EAAs)] seems to be optimal for the maximal stimulation of MPS in exercised and rested muscle of older adults.
To our knowledge, only one study has investigated the dose response of MPS to ingested protein in young adults (4). In that study (4), 20 g egg protein (equivalent to ∼8.6 g EAAs) was sufficient for the maximal stimulation of mixed MPS in ∼85-kg resistance-trained, young men. Instead of further stimulating postexercise MPS rates, 40 g egg protein stimulated a marked increase in whole-body leucine oxidation rates (4). To our knowledge, no study to date has examined the dose response of MPS after whey protein ingestion in exercised and rested muscle of resistance-trained, young adults.
All previous studies have measured the dose-response relation of MPS to ingested protein with participants in the overnight fasted state (4, 6, 8–11). To increase practical application to clinical nutrition practice, it is important that future studies investigate the dose response of MPS to ingested protein in the postabsorptive state. We showed that acute resistance exercise potentiated rates of MPS already stimulated by a protein-rich breakfast (12). Thus, the exercise-induced potentiation of MPS can be detected when exercise is performed only 1 h after food intake. Moreover, resistance exercise primarily stimulates the synthesis of myofibrillar proteins that are associated with muscle hypertrophy (13). Therefore, it also is important to characterize the dose-response relation of myofibrillar rather than mixed MPS rates and ingested doses of protein.
The purpose of the current study was to characterize the dose-response relation of postabsorptive rates of myofibrillar MPS to increasing amounts of whey protein at rest and after exercise in resistance-trained, young men. Because exercise is typically performed 2–3 h after consumption of a meal, we measured the postabsorptive response of MPS to varying doses of whey protein ingested 3 h subsequent to the consumption of a breakfast meal that contained ∼45 g protein. Because 10 g EAAs at rest (14) and 20 g egg protein after exercise (4) were optimal for the maximal stimulation of MPS in young adults, we hypothesized that 20 g whey protein (∼10 g EAAs) would be sufficient for the maximal stimulation of myofibrillar-MPS rates at rest and after resistance exercise in trained, young men.
SUBJECTS AND METHODS
Participants and ethical approval
Forty-eight healthy, active men with ≥6 mo previous recreational weight-lifting experience volunteered to participate in this study. Hence, participants were resistance-trained but did not compete in sport at a professional level. The rationale for selecting resistance-trained participants for this study was to provide a comparison with a published dose-response study in young adult men (4). Procedures followed were in accordance with the ethical standards of the National Research Ethics Service ethics board, Black Country, Birmingham (Research Ethics Committee number: 08/H1202/131) and the Helsinki Declaration of 1975 as revised in 1983. Written informed consent was provided by all participants before beginning the study. All participants were deemed healthy on the basis of their responses to a routine medical screening questionnaire. Participant characteristics within each experimental group are shown in Table 1.
TABLE 1
Participant characteristics within each experimental group1
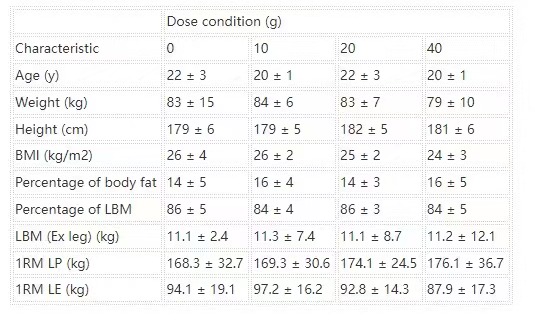
All values are means ± SDs. n = 12 in each group. Data were analyzed by using a 1-factor (dose of protein) ANOVA. No group difference was observed for any participant characteristic. Ex leg, exercised leg; LBM, lean body mass; LE, leg extension; LP, leg press; 1RM, one-repetition maximum.
Study design
This parallel research design, single-blind study was designed to determine the postabsorptive response of MPS to the ingestion of varying doses of whey protein in rested and exercised leg muscle (Figure 1). Each volunteer was assigned to 1 of 4 groups. Subsequent to a standardized breakfast, each volunteer performed an intense, unilateral leg-resistance exercise bout before ingesting a 500-mL solution that contained 0, 10, 20, or 40 g whey protein isolate (Lucozade; GlaxoSmithKline). Myofibrillar MPS, whole-body phenylalanine oxidation, and urea production rates were determined over a 4-h recovery period by combining muscle biopsies and tracer infusion of labeled phenylalanine and urea (see Calculations).
Schematic diagram of the infusion protocol. A baseline blood sample was collected before participants consumed an energy-rich, high-protein breakfast. A bout of unilateral leg-resistance Ex was performed 3 h after breakfast. Muscle biopsies (vastus lateralis) were collected from exercised and rested legs immediately after Ex and 4 h post-Ex. A drink that contained 0, 10, 20, or 40 g whey protein was ingested immediately after collection of the first set of biopsies. Multiple blood samples were collected throughout the protocol. Ex, exercise.
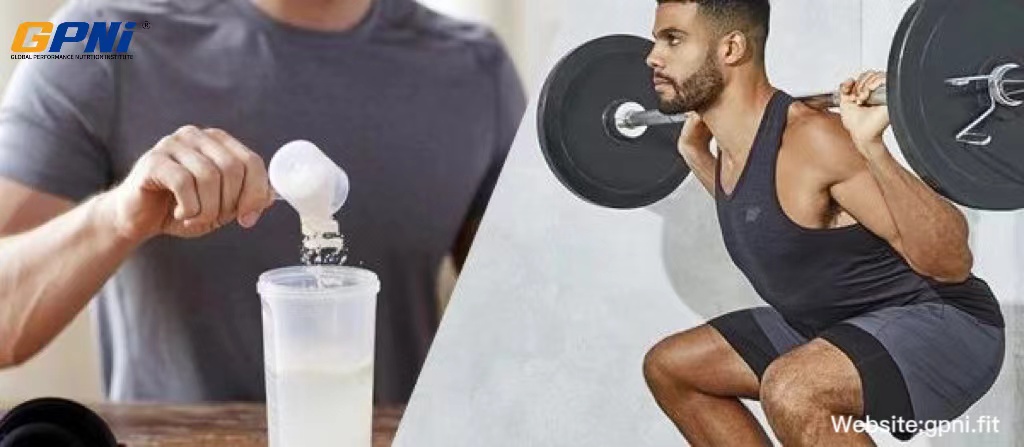
Pretesting
Body composition
Whole-body composition and leg lean mass were assessed by using dual-energy X-ray absorptiometry (Hologic Discovery W; Hologic Inc).
Maximum strength testing
At least 6 d and <2 wk before the experimental trial protocol, the maximum strength for leg-press and -extension exercises was determined. Initially, one-repetition maximum (1RM) was estimated according to a procedure modified from that of Mayhew et al (15). The allocated leg to be exercised was counterbalanced across dose conditions. When possible, groups were matched for the lean mass of their exercising leg (as determined by using dual-energy X-ray absorptiometry) and the 1RM achieved on the leg press and extension (Table 1).
Diet and physical activity control
One week before the experimental trial, participants completed a questionnaire of food preferences and a 3-d diet diary (2 weekdays and 1 weekend day) that represented their habitual daily intakes. The average energy intake (3197 ± 227 kcal) and macronutrient composition (protein: 2.3 ± 0.1 g · kg−1 body mass · d−1; carbohydrate: 4.5 ± 0.2 g · kg−1 body mass · d−1; fat: 1.3 ± 0.1 g · g−1 body mass · d−1) from the 3-d diet diary was used to calculate the participant’s diet before the experimental trial. Food parcels, which matched each participant’s habitual energy and macronutrient intakes, were supplied for 48 h before the experimental trial. Participants were instructed to consume only food and drink sources provided by investigators and to consume their final meal no later than 2000 on the evening before the experimental protocol. Analyses of the diet diary and food prescription were performed by using a commercially available software program (Wisp v3; Tinuviel Software). All participants were instructed to refrain from physical exercise for 48 h before the experimental trial.
Infusion trial
Protocol
Details of the infusion trial are shown in Figure 1. Participants reported to the Human Performance Laboratory by car, public transportation, or slow walking at ∼0615 after a 10-h overnight fast. Standard measures of height and body mass were taken. A cannula was inserted into a forearm vein and connected with a 3-way stopcock before a basal blood sample (∼12 mL) was drawn. Subjects consumed a standardized energy-rich (7 ± <1 kcal/kg body mass), high-protein (30 ± less than 1% of energy from protein, 50 ± less than 1% of energy from carbohydrate, and 20 ± <1% of energy from fat) breakfast. For the remainder of the protocol, the forearm cannula was used to infuse stable isotopic tracers. Approximately 2 min after completion of breakfast, a primed, continuous infusion (prime: ∼88 μmol/kg; infusion: ∼0.227 μmol · kg−1 · min−1) of 15N2 urea (Cambridge Isotope Laboratories) was administered in the forearm vein. A primed, continuous infusion (prime: 2.0 μmol/kg; infusion: ∼0.05 μmol · kg−1 · min−1) of L-[ring-13C6]phenylalanine (Cambridge Isotope Laboratories) was started 1 h 45 min after breakfast. A hand or wrist vein of the contralateral arm was then cannulated and heated (∼55°C) for frequent arterialized blood sampling throughout the remainder of the protocol.
Three hours after breakfast, participants performed a single bout of a heavy-load, high volume, unilateral leg-resistance exercise that consisted of 8 sets × 10 repetitions on the leg-press and -extension machines (Cybex VR3; Cybex International). Both exercises were performed at 80% 1RM with 2-min rest intervals between sets. In the event that a participant was unable to complete a set at the desired workload, the workload was reduced by 4.5 kg. The exercise routine took ∼45 min to complete.
As soon after exercise completion as possible (5 ± 1 min), muscle biopsies were collected from the vastus lateralis muscle of exercised and nonexercised (rested) legs. Immediately after biopsy collection, participants consumed a single bolus of a whey protein–containing drink. Participants were encouraged to consume beverages within 2 min. All drinks were matched for flavor (vanilla) and appearance. The amino acid content of the whey protein drink was [in the percentage of content (wt:wt)]: alanine, 5.2%; arginine, 2.2%; asparate, 11.4%; cysteine, 2.3%; glutamine, 18.8%; glycine, 1.5%; histamine, 1.8%; isoleucine, 6.7%; leucine 11.0%; lysine, 10.0%; methionine, 2.3%; phenylalanine, 3.1%; proline, 5.7%; serine, 4.8%; threonine, 7.0%; tryptophan, 1.5%; tyrosine, 2.7%; and valine, 6.1%. Hence, ingested doses of whey protein (0, 10, 20, and 40 g) consisted of 0, 5, 10, and 20 g EAAs, respectively. To minimize the disturbance in isotopic equilibrium, drinks were initially enriched to 6.5% with phenylalanine tracer according to the measured phenylalanine content of the whey protein. The initial analysis revealed that plasma enrichment increased transiently in some participants in the 40 g whey protein condition (40WP). Hence, the amount of labeled phenylalanine added to the drink was reduced to 6%. After drink ingestion, participants rested in the supine position before muscle biopsies were collected from exercised and nonexercised legs 4 h after drink ingestion.
Muscle biopsies and blood sampling
A total of 4 muscle biopsies (2 from each leg) were collected from the lateral portion of the vastus lateralis by using a 5-mm Bergstrom biopsy needle. Before the exercise bout, under local anesthetic (1% lidocaine), the lateral portion of exercised and nonexercised thighs were prepared for extraction of the tissue biopsy sample. Incisions were made before exercise to enable tissue samples to be collected as quickly as possible after exercise. On immediate completion of exercise, biopsies (exercised muscle first and nonexercised muscle second) were collected ∼10–15 cm above the knee. Bilateral biopsies were collected 4 h after drink ingestion ≥1–2 cm proximal to the previous incisions to minimize the impact of local inflammation from previous biopsy samples. All tissue samples were quickly rinsed with ice-cold saline, blotted dry, and divided into 3–4 pieces before being frozen in liquid nitrogen and stored at −80°C for additional processing.
Arterialized blood samples from a heated wrist vein were collected at rest, after breakfast, and at regular intervals after exercise. Blood samples were collected either into evacuated tubes containing K3EDTA and lithium heparin or into serum separator evacuated tubes (Becton Dickinson). Prechilled evacuated tubes containing K3EDTA and lithium heparin were temporarily placed on ice until centrifugation at 1500 × g for 15 min at 4°C. Serum-separator evacuated tubes were allowed to clot for 30 min before centrifugation at 1500 × g for 15 min at 4°C. Aliquots of plasma and serum were frozen at −80°C until later analysis.
Analytic procedures
Blood analyses
Plasma glucose and urea concentrations were analyzed with the use of an ILAB automated analyzer (Instrumentation Laboratory). Serum insulin was analyzed with the use of a commercially available ELISA (IBL International).
The arterial plasma L-[ring-13C6] phenylalanine and L-[ring– 13C6] tyrosine tracer-to-tracee ratio (t:T) was determined using electron-ionization gas chromatography–mass spectrometry (model 5973; Hewlett-Packard) as described previously (16). Briefly, 50% acetic acid was added to plasma samples before purification over cation-exchange columns (Dowex 50W-X8-200; Sigma-Aldrich). Amino acids were derivatized to their N–tert-butyldimethyl-silyl-N-methyltrifluoracetamide derivative. 13C enrichment was determined by using selected ion monitoring of masses 234 and 240 for unlabeled and labeled phenylalanine, respectively, and 466 and 472 for unlabeled and labeled tyrosine, respectively. Appropriate corrections were made for overlapping spectra contributing to the t:T. Simultaneously, plasma was analyzed for phenylalanine and leucine concentrations by using the internal standard method combined with gas chromatography–mass spectrometry analysis, as described previously (17).
A blood sample analysis for 15N2 urea enrichments also was performed following procedures described previously (18). 15N2 labeling was determined by using a selected ion monitoring of masses 231 and 233 for unlabeled and labeled urea.
Muscle analyses
Muscle tissue was analyzed for enrichment of L-[ring-13C6] phenylalanine in the bound myofibrillar protein fraction as described previously (17) by using a protocol adapted from Wilkinson et al (19). Briefly, ∼70 mg of muscle tissue was homogenized with a polytetrafluoroethylene pestle in 10 μL ice-cold homogenizing buffer (0.1 mmol KCl/L , 50 mmol tris/L, 5 mmol MgCl/L, 1 mmol EDTA/L, 10 mmol β-glycerophosphate/L, 50 mmol aF/L, and 1.5% bovine serum albumin; pH 7.5). The homogenate was centrifuged at 1000 × g for 10 min at 4°C. The pellet that remained from the original 1000 × g spin was washed twice with homogenization buffer. The myofibrillar fraction was separated from any collagen by dissolving in 0.3 mol NaCl/L, removing the supernatant fluid, and precipitating proteins with 1.0 mol perchloric acid/L. Myofibrillar fractions were washed once with 95% ethanol before being hydrolyzed overnight at 110°C in 0.1 mol HCL/L Dowex 50W X8 200 (Sigma Aldrich), and constituent amino acids were purified on cation-exchange columns (Dowex 50W X8 200; Sigma-Aldrich). Amino acids, which were liberated from myofibrillar fractions, were converted to their N-acetyl-n-propyl ester derivative, and phenylalanine labeling was determined by using gas chromatography–combustion–isotope-ratio mass spectrometry (Delta-plus XL; Thermofinnigan) with ions monitored at 44/45 for unlabeled and labeled carbon dioxide, respectively.
Calculations
Myofibrillar MPS
The fractional synthesis rate (FSR) of the myofibrillar protein fraction was calculated from the incorporation of phenylalanine into protein by using the standard precursor-product model as follows:
where ΔEm is the change in bound-protein enrichment between 2 biopsy samples, Ep is the precursor enrichment, and t is the time interval between muscle biopsies.
The FSR was calculated with the arterialized blood precursor defined as the AUC for plasma enrichments of labeled phenylalanine over the 4-h incorporation period. The AUC was chosen to minimize the influence of the transiently disturbed isotopic equilibrium experienced after drink ingestion in the 40WP (20). Given that plasma enrichments of labeled phenylalanine are higher than muscle intracellular enrichments of labeled phenylalanine, calculated FSR values may be considered to be low compared with previous studies that calculated the myofibrillar FSR by using muscle intracellular enrichments of labeled phenylalanine.
Endogenous urea production rates
The rate of urea production over time (μmol · h−1 · kg−1 body mass) was calculated as described previously (21) by using the following formula:
where i is the infusion rate of the urea tracer (μmol · h−1 · kg−1 body mass) and Eup and Eui are enrichments of urea in plasma and infusate, respectively.
Phenylalanine oxidation rates
The rate of phenylalanine oxidation (Qpt) was calculated by using the phenylalanine balance model described previously (22). In brief, whole-body phenylalanine oxidation (Qpt) was estimated from the conversion (hydroxylation) of L-[ring-13C6]phenylalanine to L-[ring-13C6]tyrosine, without the necessity to measure 13CO2 enrichment in breath gases (23). The rate of hydroxylation was calculated by using the following formula:
where Pt/Pp (=0.73) is the molar ratio of fluxes of tyrosine and phenylalanine arising from protein catabolism, and Qp equals the rate of disappearance of phenylalanine under steady state conditions. Qp (in μmol · kg−1 · h−1) was calculated as the infusion rate of 13C6 phenylalanine (∼0.05 μmol · kg−1 · min−1) multiplied by the measured enrichment of the phenylalanine infusate (t:T) divided by the phenylalanine enrichment of plasma (t:T) minus 1. Ep and Et are the L-[ring-13C6]phenylalanine and L-[ring– 13C6]tyrosine enrichments (expressed as t:T), respectively. I is the infusion rate (μmol · kg−1 · min−1).
In addition, the AUC for blood metabolites and urea production rates was calculated over the 4-h period after drink ingestion in each dose condition. Because mean 13C6 phenylalanine enrichments were briefly (∼15 min) increased after protein ingestion in the 40WP, the calculation of the AUC for phenylalanine oxidation rates did not include the initial 30 min after drink ingestion in each dose condition. The AUC is routinely used to detect differences across multiple time points (24). All variables that were expressed as the AUC over baseline were calculated with the baseline set as the value measured immediately before drink ingestion.
Data presentation and statistical analyses
A priori sample-size calculations (made with GPower 3.0.8 software), on the basis of previously published data with similar participant characteristics, determined that 12 subjects/group would be necessary to detect a statistical difference between dose conditions given an estimated effect size of 0.53, a 1-β error probability of 0.8, and a P value significance level <0.05.
All statistical tests were analyzed with SPSS version 18.0 software (SPSS Inc). Differences were considered significant if the probability of chance occurrence (α) was <0.05. All data are expressed as means ± SEMs unless otherwise stated.
A general linear model 2-factor ANOVA with repeated measures was used to analyze myofibrillar MPS rates (within-subject factor was the rested or exercised muscle; the between-subject factor was the dose of whey protein). Because of the significant main effect of dose, a 1-factor ANOVA and Bonferroni’s post hoc test were run on rested and exercised muscles combined to determine differences between doses. If a dose × muscle interaction was detected, differences in means were determined by using Bonferroni’s post hoc test.
A 2-factor ANOVA with repeated measures was used to analyze blood insulin, amino acids, and urea concentrations, whole-body urea production rates and phenylalanine and tyrosine enrichments (within-subject factor was the time point; the between-subject factor was the dose of whey protein for each measurement). If a dose × time-point interaction was detected, differences in means were determined by using Bonferroni’s post hoc test. One-factor ANOVA followed by Bonferroni’s post hoc test were used to compare AUCs of relevant variables between dose conditions.
RESULTS
Dose-response changes in glucose and insulin concentrations
The baseline plasma glucose concentration was similar for all conditions, and a similar (∼20%) increase in glucose concentration after breakfast was followed by a return to baseline 2 h later in each drink condition (data not shown).
The baseline insulin concentration was not different between conditions. Insulin concentrations markedly increased (P < 0.05) in response to breakfast, with no differences between conditions. Insulin returned to baseline values 30 min after exercise in the 0 g whey protein condition (0WP) and remained stable for the entire recovery period. Insulin peaked 30 min after whey protein ingestion and remained elevated above predrink amounts for 60 [10 g whey protein condition (10WP) and 20 g whey protein condition (20WP)] and 90 min (40WP), respectively. The insulin AUC over 4 h after drink ingestion in the 40WP was greater than in the 0WP, 10WP, and 20WP (P < 0.05), and the insulin AUC for the 20WP was greater than for the 0WP (P < 0.05; Figure 2).
FIGURE 2.
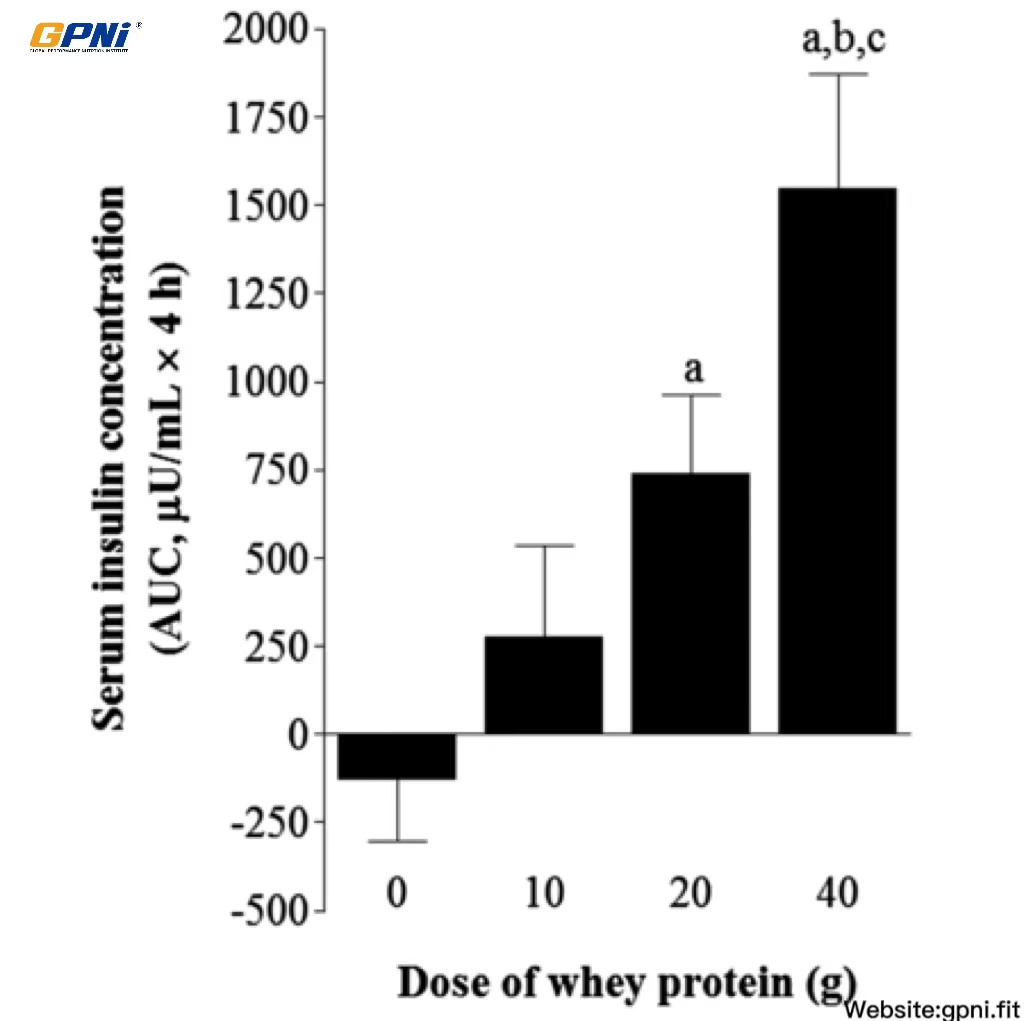
Mean (±SEM) area under the serum insulin concentration curve after postexercise ingestion of increasing doses of whey protein. Data were analyzed by using a 1-factor (dose of protein) ANOVA to test for differences between conditions. Differences in means were analyzed by using Bonferroni’s post hoc test. aSignificantly >0WP; bsignificantly >10WP; csignificantly >20WP (all P < 0.05; n = 12). 0WP, 0 g whey protein condition; 10WP, 10 g whey protein condition; 20WP, 20 g whey protein condition.
Tracer enrichments
The time course of plasma L-[ring-13C6]phenylalanine and L-[ring-13C6]tyrosine enrichments is shown in Figure 3. An initial steady state in L-[ring-13C6]phenylalanine enrichment was achieved 2 h after the start of infusion. Despite attempts to enrich the drink bolus to ∼6% enrichment, 13C6 phenylalanine enrichments were briefly (∼30 min) increased after protein ingestion in the 40WP. Thereafter, 13C6 phenylalanine enrichments returned to a steady state and remained constant for the remaining 195 min of the infusion in the 40WP. Linear regression analysis indicated that, with the exception of the blood sample collected 15 min after drink ingestion, slopes of plasma L-[ring-13C]phenylalanine enrichments over time were not significantly different from zero (P > 0.05) in all dose conditions. This information suggested that plasma enrichments had reached a plateau, and participants were at an isotopic steady state during the incorporation period. A similar pattern was observed for 13C6 tyrosine enrichment. The AUC for L-[ring-13C6]phenylalanine and L-[ring-13C6]tyrosine enrichments over 4 h after drink ingestion was similar between dose conditions.
FIGURE 3.
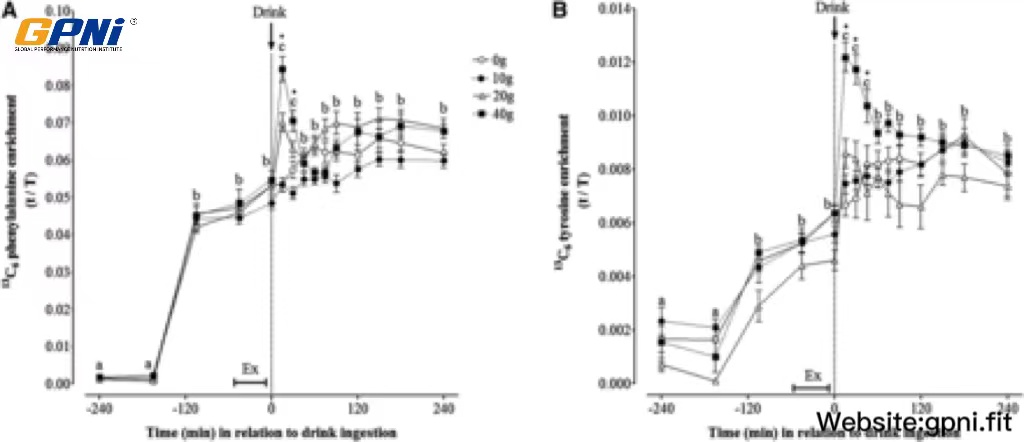
Mean (±SEM) plasma 13C6 phenylalanine (A) and 13C6 tyrosine (B) enrichments before and after postexercise ingestion of increasing doses of whey protein. Data were analyzed by using 2-factor (dose of whey protein × time point) ANOVA with repeated measures. For both 13C6 phenylalanine and 13C6 tyrosine enrichments, main effects for time and whey protein and a significant time × protein interaction were observed (all P < 0.05). Differences in means were determined by using Bonferroni’s post hoc test. Means denoted by different letters were significantly different from each other for all groups combined. *40WP >20WP, 10WP, and 0WP. Because of technical difficulties, data are expressed for n = 12 (0WP), n = 12 (10WP), n = 10 (20WP), and n = 11 (40WP). Ex, exercise; t/T, tracer-to-tracee ratio; 0WP, 0 g whey protein condition; 10WP, 10 g whey protein condition; 20WP, 20 g whey protein condition; 40WP, 40 g whey protein condition.
Dose-response changes in amino acid concentrations
The time-course of plasma phenylalanine and leucine concentrations in response to the postexercise ingestion of increasing doses of whey protein is shown in Figure 4. Amino acid concentrations were increased after breakfast (∼15 and ∼20% above baseline for phenylalanine and leucine, respectively; P < 0.05) to similar extents in all dose conditions. Exercise reduced phenylalanine and leucine concentrations to baseline concentrations in all drink conditions, with no change thereafter in 0WP. Phenylalanine and leucine concentrations increased rapidly after whey protein ingestion and peaked at either 30 min (10WP and 20WP) or 60 min (40WP) after drink ingestion. Leucine concentrations followed a dose-dependent, hierarchical pattern (ie, 40WP >20WP >10WP >0WP; P < 0.05) when expressed as the AUC over the 4-h postdrink period.
FIGURE 4.
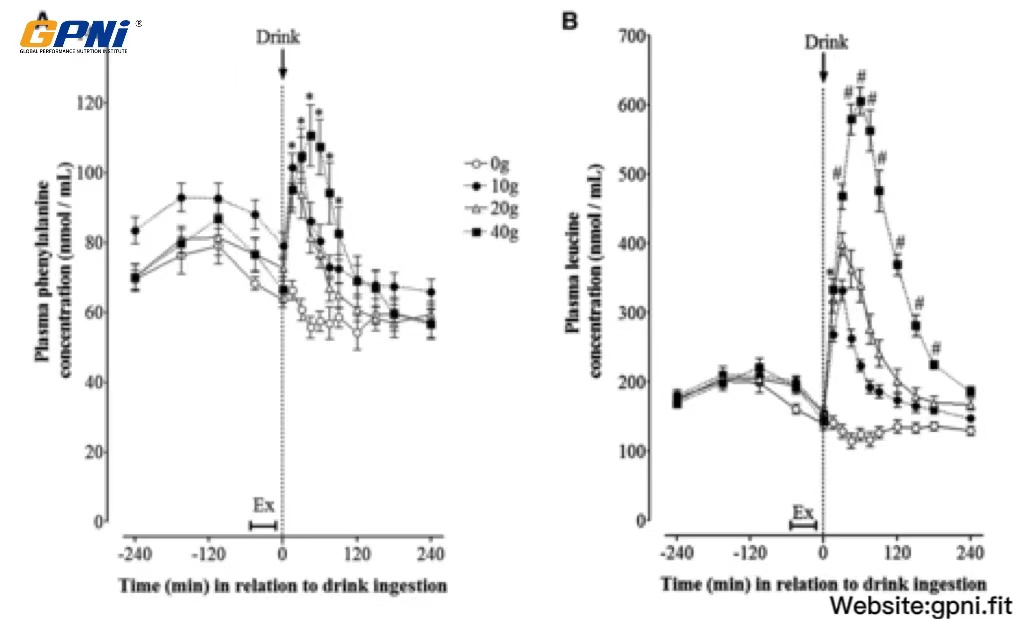
Mean (±SEM) plasma phenylalanine (A) and leucine (B) concentrations before and after postexercise ingestion of increasing doses of whey protein displayed as an overall time course. Data were analyzed by using 2-factor (dose of whey protein × time point) ANOVA with repeated measures. For both phenylalanine and leucine concentrations, main effects for time and whey protein and a significant time × protein interaction were observed (all P < 0.05). Differences in means were determined by using Bonferroni’s post hoc test. *40WP, 20WP, and 10WP >0WP; #40WP >20WP, 10WP, and 0WP (all P < 0.05, n = 12). Ex, exercise; 0WP, 0 g whey protein condition; 10WP, 10 g whey protein condition; 20WP, 20 g whey protein condition; 40WP, 40 g whey protein condition.
Dose-response changes in whole-body phenylalanine oxidation rates
The AUC of whole body phenylalanine oxidation rate in 0WP, 10WP, 20WP, and 40WP is shown in Figure 5. No differences in phenylalanine oxidation rates were observed between the 0WP, 10WP, and 20WP. The phenylalanine oxidation rate in the 40WP was greater than in all other conditions (P < 0.05).
FIGURE 5.
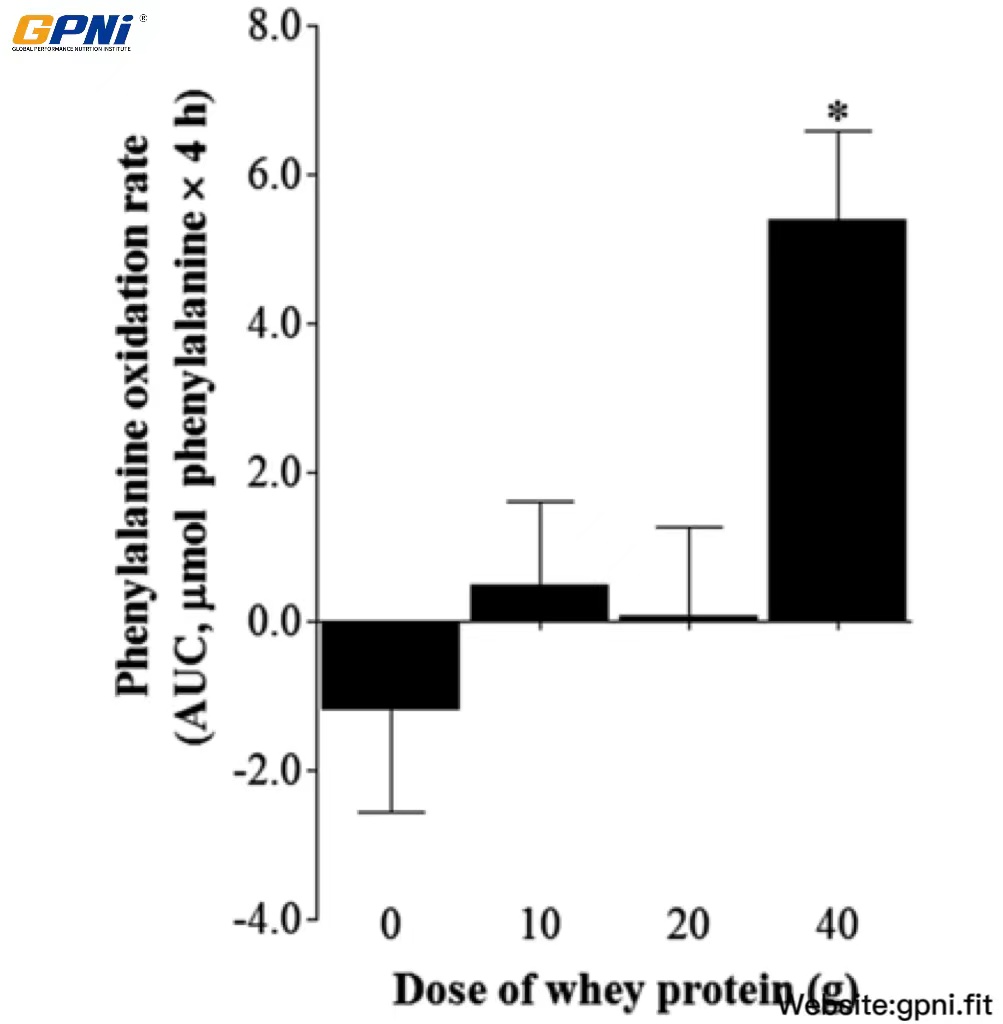
Mean (±SEM) whole-body phenylalanine oxidation rates after postexercise ingestion of increasing doses of whey protein expressed as the AUC. The calculation of the AUC for phenylalanine oxidation rates did not include the initial 30 min after drink ingestion in each dose condition. Data were analyzed by using a 1-factor (dose of protein) ANOVA to test for differences between conditions. A main effect of whey protein dose was observed (P < 0.05). Differences in means were analyzed by using Bonferroni’s post hoc test. *Significantly >0WP, 10WP, and 20WP (P < 0.05; n = 12). 0WP, 0 g whey protein condition; 10WP, 10 g whey protein condition; 20WP, 20 g whey protein condition.
Dose-response changes in whole-body urea production rates and plasma concentrations
The AUC of whole-body urea production rates and plasma concentrations in response to the postexercise ingestion of different doses of whey protein is shown in Figure 6. Urea production after whey protein ingestion (10WP, 20WP, and 40WP) was greater than in the 0WP (P < 0.05), and the urea production AUC for the 40WP was significantly greater than for the 10WP (P < 0.05) and tended (P = 0.075) to be greater than for the 20WP. The plasma urea concentration in the 40WP was greater than in the 0WP, 10WP, and 20WP (P < 0.05), and the plasma urea concentration in the 20WP and 10WP was greater than in the 0WP (P < 0.05).
FIGURE 6.
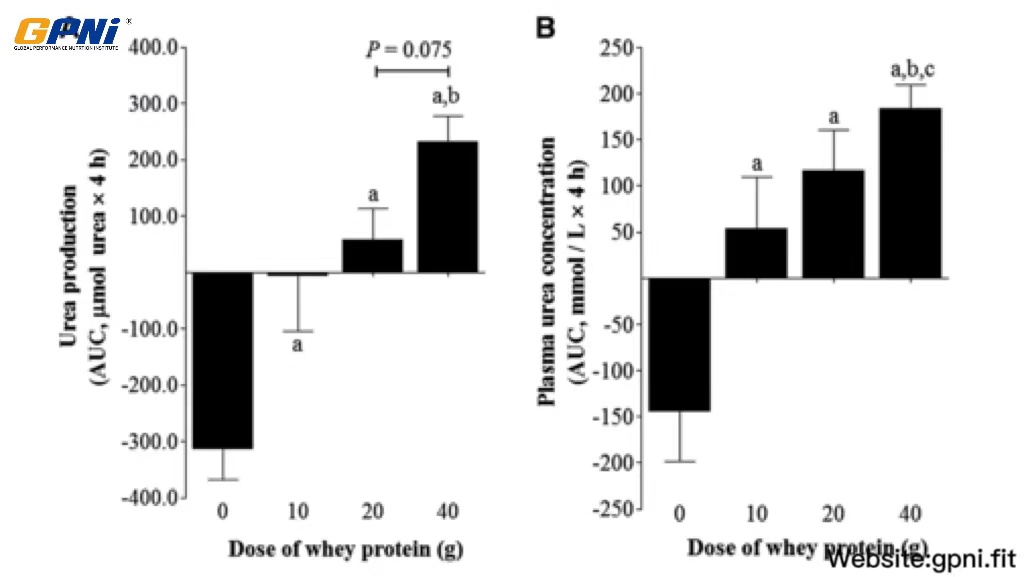
Mean (±SEM) whole-body urea production rates (A) and concentrations (B) expressed as the AUC after postexercise ingestion of increasing doses of whey protein. Data were analyzed by using a 1-factor (dose of protein) ANOVA to test for differences between conditions. A main effect of the whey protein dose was observed for both data sets (P < 0.05). Differences in means were analyzed using Bonferroni’s post hoc test. aSignificantly >0WP; bsignificantly >10WP; csignificantly >20WP (all P < 0.05, n = 12). 0WP, 0 g whey protein condition; 10WP, 10 g whey protein condition; 20WP, 20 g whey protein condition.
Dose-response changes in myofibrillar MPS rates
Overall, across all dose conditions combined, the myofibrillar FSR was greater in the exercised than in nonexercised muscle (main effect of muscle, P < 0.05; Figure 7). Although no dose × muscle interaction was detected (P = 0.437), a main effect of dose was observed across both muscles (rested and exercised) combined (P < 0.05). The myofibrillar FSR was increased (P < 0.05) above the 0WP (0.041 ± 0.015%/h) by ∼49% and ∼56% in the 20WP and 40WP, respectively, whereas no difference was observed between the 0WP and 10WP (P > 0.05). In addition, the myofibrillar FSR was increased (P < 0.05) above the 10WP by ∼22% and ∼28% in the 20WP and 40WP, respectively. No difference in myofibrillar MPS was observed between the 20WP and 40WP (P > 0.05).
FIGURE 7.
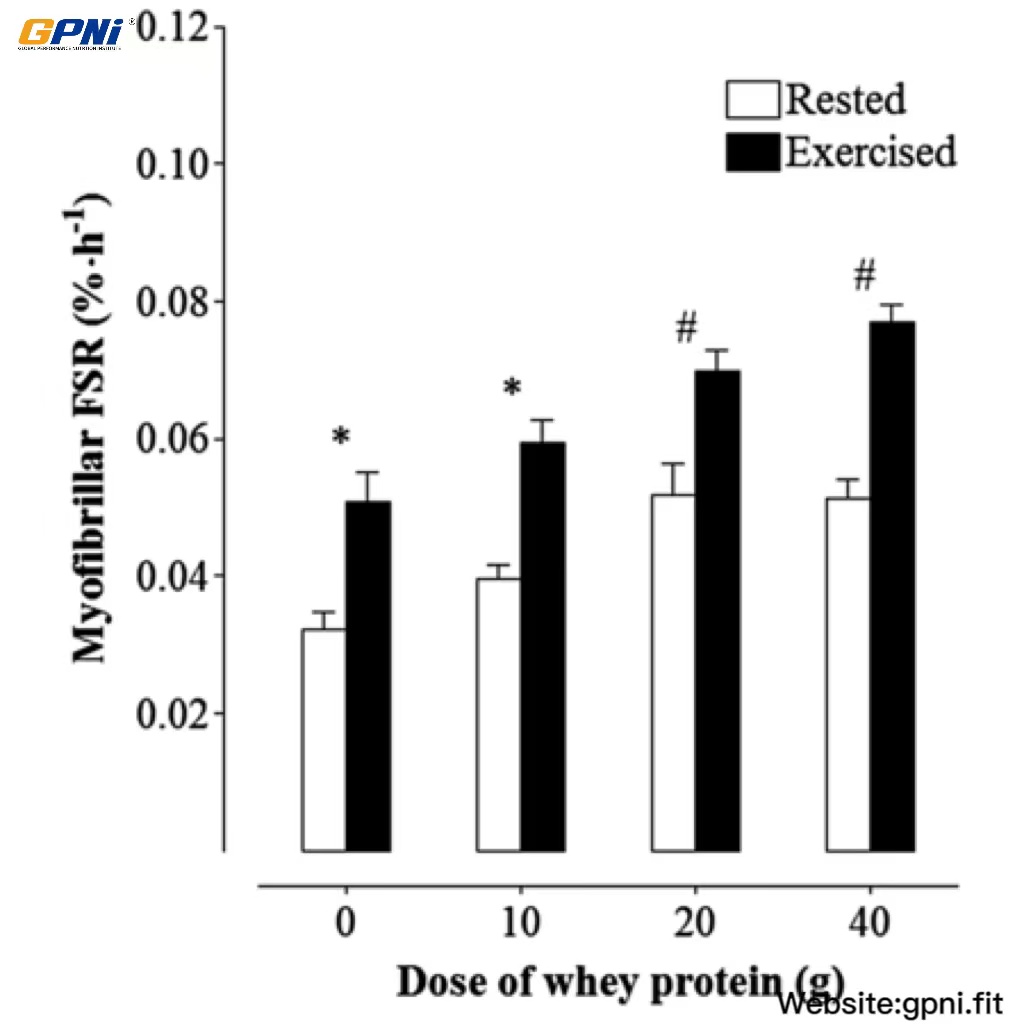
Mean (±SEM) myofibrillar muscle protein synthesis rates in response to the ingestion of varying doses of whey protein in exercise and rested muscles. The precursor was defined as the plasma phenylalanine-enrichment AUC over the 4-h incorporation period. Data were analyzed by using a 2-factor (within-subject factor was rested or exercised muscle; between-subject factor was the dose of whey protein) ANOVA with repeated measures. There were main effects for the whey protein dose (P < 0.05) and muscle (P < 0.05); however, no dose × muscle interaction was detected (P = 0.44). Differences in means for both rested and exercised muscles combined were analyzed by using Bonferroni’s post hoc test. *,#Means with different symbols were significantly different from each other (P < 0.05; n = 12). FSR, fractional synthesis rate.
DISCUSSION
Because exercise training is typically performed in the fed state, we measured postabsorptive rates of myofibrillar MPS in response to the ingestion of a 0-, 10-, 20-, or 40-g dose of whey protein isolate at rest and after resistance exercise in trained, young men. The postabsorptive ingestion of moderate (20 g) and high (40 g) doses of whey protein stimulated a greater response of myofibrillar MPS than with the low (10 g) dose of whey protein. However, myofibrillar MPS reached an upper limit with the ingestion of 20 g whey protein. Hence, in this population of ∼80-kg trained, young men, 40 g whey protein failed to further augment postabsorptive rates of myofibrillar MPS and, instead, elicited a marked increase in the rate of indicators of whole-body amino acid catabolism; the increase in amino acid oxidation was markedly greater with the ingestion of 40 g whey protein, and ureagenesis was greater with the ingestion of 20–40 g whey protein.
Past (4, 14, 25) and current data have supported the notion that an upper limit to the response of MPS to protein ingestion exists in young men, seemingly independent of protein source. We showed that the postabsorptive response of myofibrillar MPS to 20 g whey protein was ∼50% greater than when a 0-g placebo dose of whey protein was ingested and ∼20% greater than when 10 g whey protein was ingested. However, no difference in myofibrillar MPS was observed between 20- and 40-g doses of whey protein. Hence, the primary novel finding of this study was that 4-h myofibrillar MPS rates, measured after (3 h 45 min) a protein-rich (∼45 g) breakfast, were maximized by ingesting a 20-g dose of whey protein. These data were consistent with previous dose-response studies in rested (14) and exercised (4) muscles. Cuthbertson et al (14) showed that the ingestion of 10 g of rapidly digested free-form EAAs (typically contained in 20 g intact protein) was sufficient for the maximal stimulation of myofibrillar MPS in rested muscles of untrained, young men. With regard to whole-food sources, a moderately sized protein meal (equivalent to 113 g lean beef, 30 g protein, and ∼10 g EAAs) was equally effective as a large-sized protein meal (340 g) serving of lean beef (90 g protein and 30 g EAAs) for the stimulation of mixed MPS (11). Accordingly, Moore et al (4) showed that ingesting a 20-g dose of egg protein after an exercise bout in the fasted state was sufficient to maximize the postexercise response of mixed MPS in ∼85-kg resistance-trained, young men. Hence, irrespective of whether a single bolus dose of amino acids is ingested in the fasted or postabsorptive state, a dose of 20 g protein (equivalent to ∼10 g EAAs) is maximally effective for stimulating MPS at rest or postexercise in 80–85-kg young men. The dose response of MPS to ingested casein or lower-quality protein, such as soy, in young adults remains unknown but likely mirrors, at least qualitatively, the EAA composition (in particular of leucine) of protein sources.
The “muscle full effect” concept (26) offers an explanation for why MPS rates were not augmented with 40 g protein in the current and past (4) studies. This concept is based on the notion that an upper limit of amino acid delivery must be achieved before muscle cells no longer use amino acids as substrate for MPS and, instead, divert amino acids toward catabolic processes (27). Both the extracellular (25) and intracellular availability of amino acids regulates the response of MPS. Changes in EAA concentrations are sensed by a leucine membrane detector that subsequently signals the activation of mammalian target of rapamycin signaling to stimulate MPS (28). Previous work reported a similar rested response of myofibrillar MPS to the ingestion of 10, 20, or 40 g EAAs, despite markedly greater peak changes in intracellular leucine concentrations after the ingestion of high (20–40 g) doses compared with a moderate (10 g) dose of EAAs (14). Accordingly, our data showed that peak changes in arterialized blood amino acid concentrations increased stepwise in relation to the whey protein dose (∼60%, ∼80%, and >200% increase above basal in the 10WP, 20WP, and 40WP, respectively); however, no additional stimulation of myofibrillar MPS was observed in the 40WP. This apparent disconnect between amino acid availability and the response of MPS to the 40-g dose of whey protein was consistent with the concept of a “muscle full effect” (26). Moreover, we (Figure 5) and others researchers (4) have shown a pronounced increase in whole-body rates of amino acid oxidation with the ingestion of 40 g protein. Indeed, in the current study, urea production rates (Figure 6A), as well as plasma urea concentrations (Figure 6B), were markedly raised with the ingestion of 40 g protein. Thus, instead of incorporation into muscle protein, the metabolic fate of excess exogenous amino acids contained in the 40WP was predominantly the oxidation or excretion as an indication that a state of amino acid excess was reached (29).
The “muscle full effect” (26) from breakfast also may affect the response of MPS to small (10 g) doses of whey protein. We administered whey protein ∼3 h 45 min after a breakfast meal that contained ∼0.55 g protein/kg. Myofibrillar MPS was restimulated by 20 g whey protein. Hence, our data suggested that, after already being filled by a high-protein breakfast meal, young muscle can again respond to EAAs ∼3 h 45 min later if sufficient protein is ingested. However, no significant stimulatory impact of a 10-g dose of whey protein was shown, despite an ∼20% greater mean response of myofibrillar MPS in the 10WP compared with 0WP. In contrast, previous studies showed that the ingestion of 10 g protein after a fasted-state resistance-exercise bout stimulated a 50% increase in mixed MPS rates than did exercise without protein ingestion (30). One explanation for these equivocal findings may be that MPS rates in the 0WP were raised by the breakfast meal, and therefore, the likelihood of detecting a difference in MPS rates between the 0WP and 10WP was reduced compared with in previous studies. In addition, a refractory response to the breakfast meal and simultaneous decreased sensitivity of skeletal muscle to hyperaminoacidemia in the current study also was possible. Alternatively, it could be argued that the results were underpowered. However, power calculations of our myofibrillar MPS dataset revealed 1-β error probabilities of 0.6 and 0.8 in exercised and rested muscle, respectively, which inferred that a moderate-to-high statistical power was achieved.
These data exhibited clear clinical implications to populations who desire muscle-mass gain or muscle maintenance. To strengthen the practical relevance of our study design to the majority of exercisers who lift weights in the fed state, we characterized the dose-response of myofibrillar MPS to ingested whey protein in both rested and exercised muscles subsequent to the consumption of a breakfast meal. In the current study, FSR values were calculated by using plasma enrichments of labeled phenylalanine and, thus, values may be considered to be low compared with in previous studies that calculated myofibrillar FSR by using muscle intracellular enrichments of labeled phenylalanine (14). Nonetheless, a 2-way repeated-measures ANOVA revealed no significant dose (0–40 g) × muscle (rested and exercised legs) interaction of myofibrillar MPS rates (P = 0.44). Thus, a post hoc comparison between dose conditions within rested and exercised muscles separately was not possible. However, intriguingly, mean postexercise myofibrillar MPS values were ∼19% higher in the 20WP than 10WP but only an additional ∼10% higher in the 40WP. An additional calculation of the 95% CI for the true difference between the 20WP and 40WP revealed that we could not rule out a 14% increase in the myofibrillar FSR with the ingestion of 40 g whey protein. Notably consistent with this observation was the previously reported ∼9% difference in the postexercise mixed MPS response between 20 and 40 g egg protein in resistance-trained men (4). Hence, for 80–85-kg resistance-trained, young men, past (31, 32) and current data suggested that a diminished return in terms of the acute stimulation of postexercise rates of MPS is gained from repeatedly ingesting >20 g protein. In contrast, the long-term physiologic impact of a 10–14% higher rate of MPS in the 40WP on muscle hypertrophy remains unknown. Furthermore, it is possible that the optimal dose of protein for maximizing MPS depends on individual muscle mass. It remains unknown whether the optimal dose of protein for the maximal stimulation of MPS differs between the 120-kg athlete and 80-kg male exerciser compared with the 60-kg female exerciser. Future studies should be designed to fully elucidate how muscle mass or the amount of muscle mass exercised influences the response of MPS to different doses of protein. It is intuitive that nutritional recommendations should be specified not only to the individual’s age but also to the lean body mass, sex, and training status of the individual.
In conclusion, the postabsorptive response of myofibrillar MPS in ∼80-kg resistance-trained, young men are dose-dependent on ingested protein, rising to an upper limit with 20 g whey protein. The ingestion of a dose of whey protein >20 g results in a diminished return in terms of stimulating myofibrillar MPS and, instead, stimulates amino acid oxidation and ureagenesis. Future clinical work is needed to characterize the maximal anabolic response of muscle, with consideration of both MPS and muscle protein breakdown, to protein ingested in meal form (2).
We thank Debbie Rankin and Robert Bagabo for their technical assistance; Gareth Wallis for his input regarding the study design; and Rebecca Randell, Adrian Hodgson, Thomas Rowlands, Sam Shepherd, Matt Cocks, and Helen Bradley for their assistance during infusion trials. We also extend our appreciation to participants for their time and effort.
The authors’ responsibilities were as follows—OCW, SRJ, LB, and KDT: designed the research project and conducted the research; OCW, SRJ, LB, KS, and KDT: wrote the manuscript; OCW and KDT: had primary responsibility for the final content of the manuscript; and all authors: analyzed data or performed statistical analyses and read and approved the manuscript. GlaxoSmithKline Nutritional Healthcare was involved in the design, analysis, and interpretation of data. None of the authors had a conflict of interest.
FOOTNOTES
Supported by GlaxoSmithKline Nutritional Healthcare (research grant to KDT).
REFERENCES
- Wolfe RR.The underappreciated role of muscle in health and disease.Am J Clin Nutr 2006;84:475-82.
- Deutz NE, Wolfe RR.Is there a maximal anabolic response to protein intake with a meal?Clin Nutr 2013;32:309-13.
Google Scholar Crossref PubMed
- Rennie MJ,Tipton KD .Protein and amino acid metabolism during and after exercise and the effects of nutrition. Annu Rev Nutr 2000;20:457-83.
Google Scholar Crossref PubMed
- Moore DR,Robinson MJ,Fry JL, Tang JE,Glover EI,Wilkinson SB,Prior T,Tarnopolsky MA,Phillips SM.Ingested protein dose response of muscle and albumin protein synthesis after resistance exercise in young men.Am J Clin Nutr 2009;89:161-8.
Google Scholar Crossref PubMed
- Pennings B,Boirie Y,Senden JM,Gijsen AP,Kuipers H,van Loon LJ.Whey protein stimulates postprandial muscle protein accretion more effectively than do casein and casein hydrolysate in older men.Am J Clin Nutr 2011;93:997-1005.
Google Scholar Crossref PubMed
- Pennings B,Groen B,de Lange A,Gijsen AP,Zorenc AH,Senden JM,van Loon LJ.Amino acid absorption and subsequent muscle protein accretion following graded intakes of whey protein in elderly men.Am J Physiol Endocrinol Metab 2012;302:E992-9.
Google Scholar Crossref PubMed
- Tang JE,Moore DR,Kujbida GW,Tarnopolsky MA,Phillips SM.Ingestion of whey hydrolysate, casein, or soy protein isolate: effects on mixed muscle protein synthesis at rest and following resistance exercise in young men.J Appl Physiol 2009;107:987-92.
Google Scholar Crossref PubMed
- Yang Y,Breen L,Burd NA,Hector AJ,Churchward-Venne TA,Josse AR,Tarnopolsky MA,Phillips SM.Resistance exercise enhances myofibrillar protein synthesis with graded intakes of whey protein in older men.Br J Nutr 2012;108:1780-8.
Google Scholar Crossref PubMed
- Yang Y,Churchward-Venne TA,Burd NA,Breen L,Tarnopolsky MA,Phillips SM.Myofibrillar protein synthesis following ingestion of soy protein isolate at rest and after resistance exercise in elderly men.Nutr Metab (Lond) 2012;9:57.
Google Scholar Crossref PubMed
- Robinson MJ, Burd NA,Breen L,Rerecich T,Yang Y,Hector AJ,Baker SK,Phillips SM.Dose-dependent responses of myofibrillar protein synthesis with beef ingestion are enhanced with resistance exercise in middle-aged men.Appl Physiol Nutr Metab 2013;38:120-5.
Google Scholar Crossref PubMed
- Symons TB, Sheffield-Moore M,Wolfe RR,Paddon-Jones D.A moderate serving of high-quality protein maximally stimulates skeletal muscle protein synthesis in young and elderly subjects.J Am Diet Assoc 2009;109:1582-6.
Google Scholar Crossref PubMed
- Witard OC,Tieland M,Beelen M,Tipton KD,van Loon LJ,Koopman R.Resistance exercise increases postprandial muscle protein synthesis in humans.Med Sci Sports Exerc 2009;41:144-54.
Google Scholar Crossref PubMed
- Moore DR, Tang JE, Burd NA, Rerecich T, Tarnopolsky MA, Phillips SM. Differential stimulation of myofibrillar and sarcoplasmic protein synthesis with protein ingestion at rest and after resistance exercise. J Physiol 2009;587:897–904.
Google Scholar Crossref PubMed
- Cuthbertson D, Smith K, Babraj J, Leese G, Waddell T, Atherton P, Wackerhage H, Taylor PM, Rennie MJ. Anabolic signaling deficits underlie amino acid resistance of wasting, aging muscle. FASEB J 2005;19:422–4.
Google Scholar Crossref PubMed
- Mayhew JL, Ball TE, Arnold TE, Bowen JC. Relative muscular endurance as a predictor of bench press in college men and women . J Appl Sports Sci Res 1992;6:200–6.Google Scholar
- Koopman R, Wagenmakers AJ, Manders RJ, Zorenc AH, Senden JM, Gorselink M, Keizer HA, van Loon LJ. Combined ingestion of protein and free leucine with carbohydrate increases postexercise muscle protein synthesis in vivo in male subjects. Am J Physiol Endocrinol Metab 2005;288:E645–53.
Google Scholar Crossref PubMed
- Breen L, Philp A, Witard OC, Jackman SR, Selby A, Smith K, Baar K, Tipton KD. The influence of carbohydrate-protein co-ingestion following endurance exercise on myofibrillar and mitochondrial protein synthesis. J Physiol 2011;589:4011–25.
Google Scholar Crossref PubMed
- Jahoor F. Reassessment of primed constant-infusion tracer method to measure urea kinetics. Am J Physiol 1987;252:E557–64.
- Wilkinson SB, Phillips SM, Atherton PJ, Patel R, Yarasheski KE, Tarnopolsky MA, Rennie MJ. Differential effects of resistance and endurance exercise in the fed state on signalling molecule phosphorylation and protein synthesis in human muscle. J Physiol 2008;586:3701–17.
Google Scholar Crossref PubMed
- Zilversmit DB. The design and analysis of isotope experiments. Am J Med 1960;29:832–48.
Google Scholar Crossref PubMed
- Jahoor F, Wolfe RR. Reassessment of primed constant-infusion tracer method to measure urea kinetics. Am J Physiol 1987;252:E557–64.
- Munro H, Fleck A. Analysis of tissues and body fluids for nitrogenous constituents. In: Munro HN, ed. Mammalian protein metabolism. New York, NY: Academic Press, 1969:423–525.
Thompson GN, Pacy PJ, Merritt H, Ford GC, Read MA, Cheng KN, Halliday D. Rapid measurement of whole body and forearm protein turnover using a [2H5]phenylalanine model. Am J Physiol 1989;256:E631–9.
- Pruessner JC, KirschbaumC, Meinlschmid G, Hellhammer DH. Two formulas for computation of the area under the curve represent measures of total hormone concentration versus time-dependent change. Psychoneuroendocrinology 2003;28:916–31.
Google Scholar Crossref PubMed
- Bohé J, Low A, Wolfe RR, Rennie MJ. Human muscle protein synthesis is modulated by extracellular, not intramuscular amino acid availability: a dose-response study. J Physiol 2003;552:315–24.
Google Scholar Crossref PubMed
- Atherton PJ, Etheridge T, Watt PW, Wilkinson D, Selby A, Rankin D, Smith K, Rennie MJ. Muscle full effect after oral protein: time-dependent concordance and discordance between human muscle protein synthesis and mTORC1 signaling. Am J Clin Nutr 2010;92:1080–8.
Google Scholar Crossref PubMed
- Bohé J, Low JF, Wolfe RR, Rennie MJ. Latency and duration of stimulation of human muscle protein synthesis during continuous infusion of amino acids. J Physiol 2001;532:575–9.
Google Scholar Crossref PubMed
- Kimball SR, Jefferson LS. Control of translation initiation through integration of signals generated by hormones, nutrients, and exercise. J Biol Chem 2010;285:29027–32.
Google Scholar Crossref PubMed
- Zello GA, Wykes LJ, Ball RO, Pencharz PB. Recent advances in methods of assessing dietary amino acid requirements for adult humans. J Nutr 1995;125:2907–15.
- Kim PL, Staron RS, Phillips SM. Fasted-state skeletal muscle protein synthesis after resistance exercise is altered with training. J Physiol 2005;568(pt 1):283–90.
- Areta JL, Burke LM, Ross ML, Camera DM, West DW, Broad EM, Jeacocke NA, Moore DR, Stellingwerff T, Phillips SM, et al. Timing and distribution of protein ingestion during prolonged recovery from resistance exercise alters myofibrillar protein synthesis. J Physiol 2013;591:2319–31.
Google Scholar Crossref PubMed
- Moore DR, Areta J, Coffey VG, Stellingwerff T, Phillips SM, Burke LM, Cleroux M, Godin JP, Hawley JA. Daytime pattern of post-exercise protein intake affects whole-body protein turnover in resistance-trained males. Nutr Metab (Lond)2012;9:91.
Google Scholar Crossref PubMed
ABBREVIATIONS
- EAAessential amino acid
- FSRfractional synthesis rate
- MPSmuscle protein synthesis
- t:Ttracer-to-tracee ratio
- 0WP0 g whey protein condition
- 1RMone-repetition maximum
- 10WP10 g whey protein condition
- 20WP20 g whey protein condition
- 40WP40 g whey protein condition.